Evaluation reveals factors reducing productivity, proposes improvements for efficient management
Closed systems for shrimp culture have been developed in recent years to mitigate some environmental issues and to reduce the risk of shrimp disease. Super-intensive culture systems using minimal exchange of rearing water are evolving and promising new aquaculture technologies that can conserve land and water resources and reduce environmental impacts. Some recent studies have tried to evaluate the optimal conditions for increasing shrimp biomass production. However, few studies have examined the balance of costs and benefits of closed-system culture under commercial production. Ways of achieving optimal economic management and addressing unforeseen problems when these systems are adopted have not been sufficiently explored.
Simulations based on numerical models can provide insight into how to achieve better management strategies. Bio-economic modeling is one such adequate approach for studying the complex interactions among the different factors that affect aquaculture production. Along these lines, there are several examples of recent studies using bioeconomic approaches that have been useful in improving strategies for shrimp farming in intensive and semi-intensive systems.
This article — adapted and summarized from the original publication (Shinji, J. et al. 2019. Bio-economic analysis of super-intensive closed shrimp farming and improvement of management plans: a case study in Japan. Fish Sci 85, 1055–1065) — describes a study that focused on the utility of such bio-economic approaches in improving management strategy for super-intensive closed culture shrimp production using the Indoor Shrimp Production System (ISPS) plant operating in Myoko City, Niigata Prefecture (Japan) as a case study.
Study setup
We used the Indoor Shrimp Production System (ISPS) shrimp plant located in Myoko City, Niigata Prefecture (Japan), as the case study site (operated by IMT Engineering Inc., Tokyo, Japan). Rearing data for eight batches of Pacific white shrimp (Litopenaeus vannamei) were provided by the operator of the aquaculture system (IMT Engineering Inc.). The ISPS is a super-intensive closed culture system that recirculates the rearing water, with virtually no water inflow and outflow between the production lanes and the outside. Environmental factors can be easily maintained, as the control of this system is easier than that of conventional aquaculture systems that are not enclosed.
Moreover, the price of the shrimp product has already been defined by cross-trading and does not change with market supply and demand, and shrimp products are generally sold out at every production cycle. We considered that this system contains few uncertain factors in terms of both farming and market conditions, making it preferable for analyzing the mechanisms of both aquacultural and economic production.
To establish a model for the purpose of optimizing production plans at the study site, we performed path analysis using structural equation modeling (SEM). This process involves screening the critical factors that determine the dynamics of shrimp production at the chosen study site by holistically analyzing the relationships among daily changes in environmental factors, rearing conditions, growth rates and mortality rates. We focused on the grow-out stage of the production process (following the nursery phase), because shrimp biomass mostly increases during this phase, and is thus the most important part of the process to target in order to achieve overall improved production efficiency. Then we established models relating to population dynamics.
Harvesting models were developed to estimate profits; harvesting is also a means of controlling the population dynamics in an aquaculture setting by reducing future dead biomass via the process of culling. Accordingly, the harvesting model includes culling as a means of circumventing risk due to mortality and improving overall yield, leading to economic gain. The economic yield can be directly calculated using the harvesting model independent of the influence of the shrimp market, because the price is fixed by cross-trading. The profit per single batch and the annual profit can be calculated based on the balance of the economic yield and the total cost required for production.
For detailed information on the case study site; data sources; concept of analysis and model specification; and simulation methodology, refer to the original publication.
Results and discussion
Many biological studies — especially those focused on physiological and pathological aspects — have strengthened and supported the expansion of commercial shrimp farming, but the importance of the final economic output of the commercial industry has been less well considered in the research field. In our study, we aimed at formulating an optimal aquaculture strategy by using bio-economic models. This approach has the advantage that it can be used to analyze complex interactions of various factors in the actual processes of commercial aquaculture production and thus has implications for the industry’s economic management. We applied the methodology to an indoor, intensive, closed culture system, a shrimp aquaculture option that is growing everywhere.
Our first analytical step using SEM evaluated aquaculture problems in an indoor closed super-intensive culture system, because it is possible that as-yet-unknown issues in rearing conditions will surface in this type of system (Fig. 1). Many studies have focused on the importance of chemical and physical environmental conditions limiting or decreasing productivity in shrimp farming; such conditions include salinity, water temperature, dissolved oxygen and nitrogenous wastes.
However, our SEM model indicated that none of these conditions functioned to decrease productivity, as most of these parameters were maintained within acceptable levels in the production lanes used in our study. The notable point in our SEM model was the predicted major effect of cumulative mortality on daily mortality. The prediction given by the SEM model was supported by our multiple regression analysis. This means that mortality leads to further mortality, and that production loss increases in a chain reaction once mortality is triggered in the population.
There is no information about the unidentified environmental factors critical to these kinds of shrimp farming systems. If the use of super-intensive closed systems is to be promoted, then we must accept that it will be essential in the future to clarify what these unidentified environmental factors are.
Simulations changing the parameters comprising population dynamics showed the importance of population management and its details. The early part of the mortality curve was sensitive to an unexpected increase in mortality. It is therefore important to control mortality by maintaining rearing conditions. Our simulation also showed the importance of growth control. Our results emphasized the serious productive loss caused by an accidental growth decrease – more than the predicted productive gain from growth improvement. Growth decrease delays the start of harvesting. This delay prolongs the farming schedule for a single run and increases the risk of production loss through mortality.
Our simulations showed the importance of controlling biomass-scale population dynamics to prevent chain-reaction-type production losses. Artificial population subtraction may be one of the most effective management methods for controlling populations. The estimated effect is high when the mortality curve is sensitive to the unexpected increase in mortality, suggesting the efficacy of using population subtraction during the critical period. Our results showed the importance of having a proper harvesting plan to achieve successful biological population management. The effects of the stocking population on production is a common interest found throughout biological and bio-economic studies in aquaculture. This is similarly shown in our results, but our finding differs in that it indicates the utility of population control as a method for preventing exponential production loss.
Our optimized harvesting plans recommended earlier and concentrated harvesting to secure economic improvement (Figs. 2-3). These plans maximize the difference between economic yield through growth and production loss through death. Optimized plans that shortened the total period required for a single culture run indicated that faster rotation led to better economic profits as well. A faster rotation plan produced a 68 percent increase in annual economic yield in return for a 26 percent increase in each running cost. Faster production rotation has often been indicated as an effective means of achieving economic improvement in commercial shrimp culture, although the details of the estimations differ.
The production plan optimized here showed the possibility of drastically improving profits. However, the percentage of shrimp belonging to the body size class that was sold at higher prices was extremely low, especially in the case of maximizing annual profit. This indicates that the economic yields from the larger and higher-priced shrimps cannot compensate for the increased costs of growth and the production losses caused by mortality during the prolonged rearing period for these larger shrimps. Actual harvesting at the study site was more similar to the harvesting plan that maximized production per batch rather than annual profit (Fig. 3). This suggests that the harvesting plan at the study site has not been optimized for economic gain. Nevertheless, the operator of the study site has tended to produce larger shrimp without considering economic loss, and it has repeated this production plan without changing its direction for at least two years.
Farm workers believe that production of the larger shrimp creates brand power. “Brand” is an attribute that is effective in increasing trading prices and product size is a factor supporting seafood brand image. The company has succeeded in establishing its own, original brand with higher prices through their original farming system. In such a case, there is often a first-mover competitive advantage.
However, it is not clear in this case whether the benefits obtained from the brand image are equivalent to, or more valuable than, the predicted opportunistic cost to maintain the brand image. As long as the plant operator believes in the brand power brought by the production of larger shrimp, there is another problem: How much production is required to maintain the brand image? This is an important point in clarifying why the plant operator repeatedly chose the present strategy and is important in relation to the plant operator’s future acceptance of suggested plans for improving economic management.
Perspectives
We used simulations based on bio-economic models to show the factors predicted to cause shrimp mortality in a super-intensive closed culture system, and we produced improved plans for maximizing profits at the study site. Our study suggests that an as-yet unidentified factor relating to cumulative mortality in the closed system is especially critical in causing death, and we propose that the identification of this factor will be an important research subject for the future.
Another important finding was that economic management itself functions as a form of shrimp population control that is effective in reducing production losses in the presence of the unidentified factor. Our analysis suggests that the company at the study site had a potential mismatch between the best economic strategies and actual production. The improved production schedule suggested here might achieve a dramatic increase in the profits of shrimp culture by earlier and more concentrated harvesting in super-intensive closed culture systems.
In conclusion, this case study has demonstrated the utility of bio-economic information in analyzing such production systems; our approach may open new possibilities for the use of bio-economic modeling, for example, to design and develop an algorithmic package to control the actual production of shrimp on site.
Now that you've reached the end of the article ...
… please consider supporting GSA’s mission to advance responsible seafood practices through education, advocacy and third-party assurances. The Advocate aims to document the evolution of responsible seafood practices and share the expansive knowledge of our vast network of contributors.
By becoming a Global Seafood Alliance member, you’re ensuring that all of the pre-competitive work we do through member benefits, resources and events can continue. Individual membership costs just $50 a year.
Not a GSA member? Join us.
Authors
-
Junpei Shinji, Ph.D.
Department of Global Agricultural Sciences
Graduate School of Agricultural and Life Sciences
The University of Tokyo
1-1-1 Yayoi, Bunkyo-ku, Tokyo, 113-8657, Japan -
Nobuyuki Yagi, Ph.D.
Department of Global Agricultural Sciences
Graduate School of Agricultural and Life Sciences
The University of Tokyo
1-1-1 Yayoi, Bunkyo-ku, Tokyo, 113-8657, Japan -
Setsuo Nohara, Ph.D.
IMT Engineering, Inc.
1-23-1 Shinjuku, Shinjuku-ku, Tokyo, 160-0022, Japan -
Marcy Wilder, Ph.D.
Corresponding author
Japan International Research Center for Agricultural Sciences
1-1 Ohwashi, Tsukuba, Ibaraki, 305-8686, Japan
[112,106,46,111,103,46,99,114,102,102,97,64,108,105,119,114,97,109]
Tagged With
Related Posts
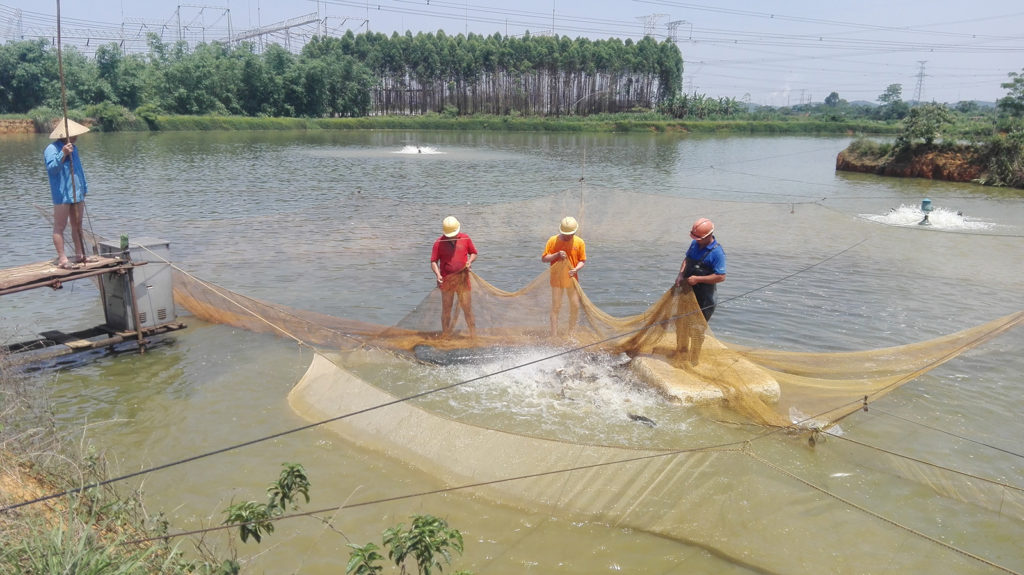
Innovation & Investment
Bio-economic modeling for improving aquaculture performance
Experience in China, the world’s largest tilapia farming country, is used to develop and calibrate a bio-economic model of intensive tilapia pond culture. It is used to simulate the impacts of climate, technical and/or economic factors on farming.
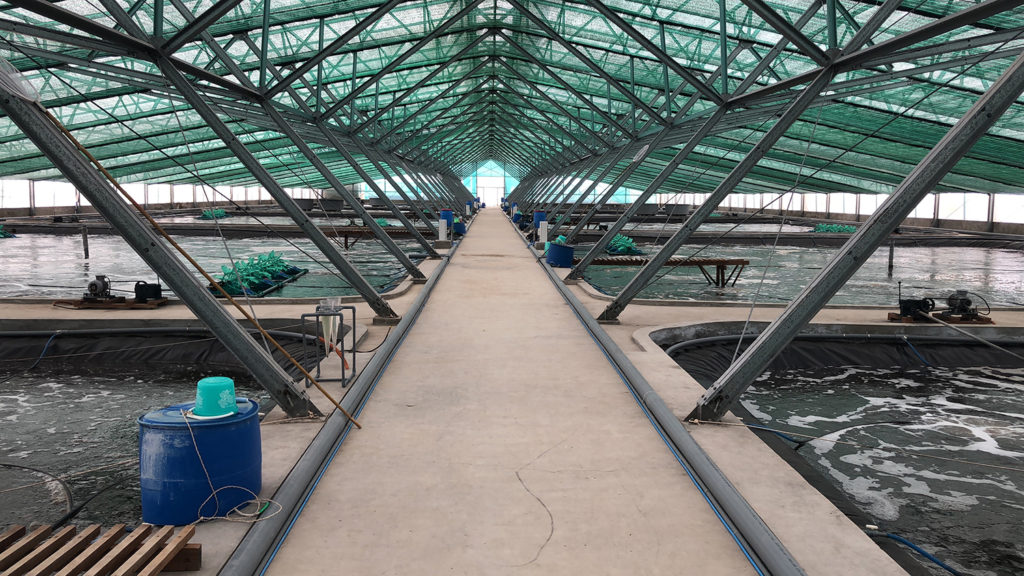
Innovation & Investment
Collaboration drives innovations in super-intensive indoor shrimp farming
A collaboration between Viet-Uc Seafood Corporation and CSIRO of Australia develops improvements in super-intensive indoor shrimp farming technology.
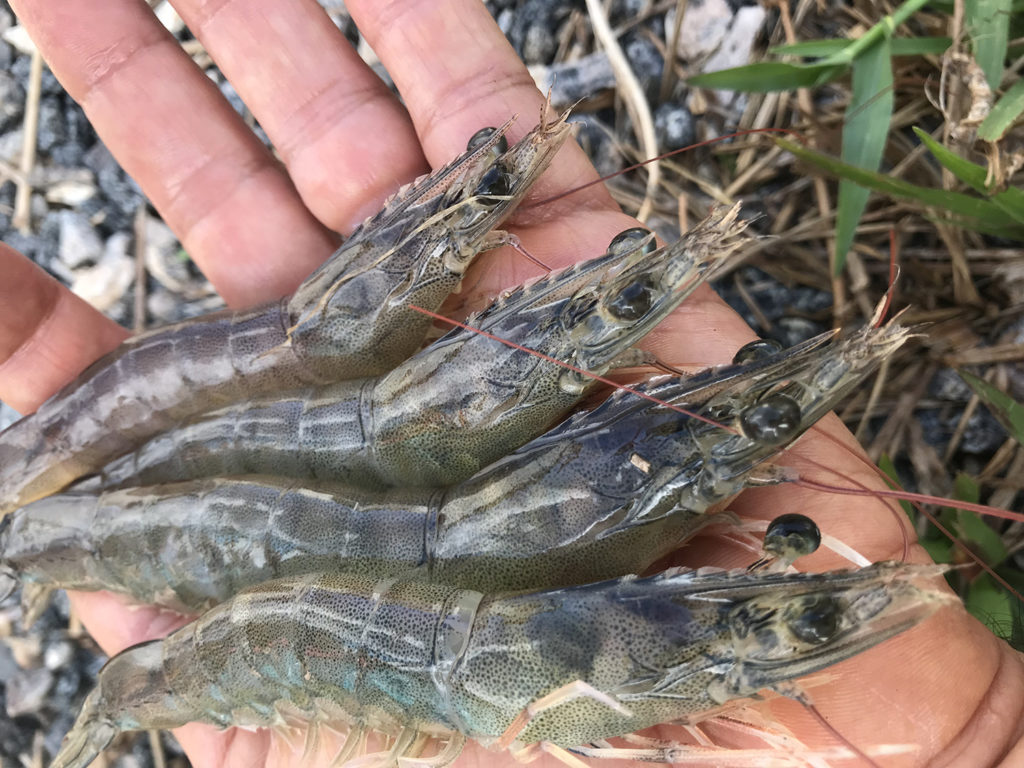
Health & Welfare
Culture of Pacific white shrimp juveniles in super-intensive conditions
Results of this study showed that L. vannamei juveniles can be cultured at high densities with adequate survival, growth and feeding efficiency, and with minimal water exchange or full water reuse through recirculation.
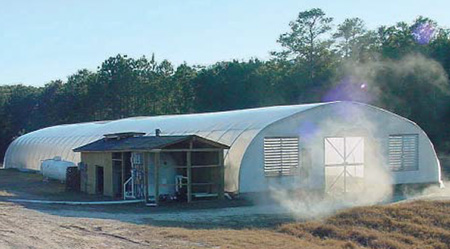
Intelligence
Greenhouse raceways: A consideration for super intensive shrimp production
Greenhouse raceways can be managed with zero to minimal water exchange, thus greatly reducing environmental impacts due to effluent discharge.